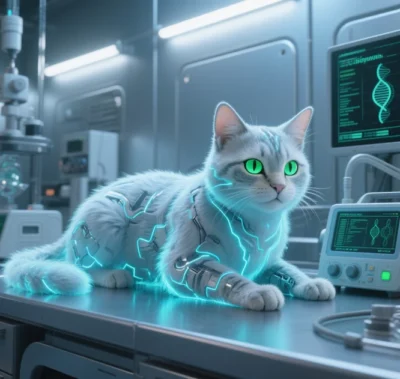
Genome Engineering and Synthetic Genomics: Technological Paradigms, Differences, and Collaborative Evolution
I. Core Definitions and Scope Comparison
- Genome Engineering
- Definition: A set of technologies for precise editing, deletion, or insertion of DNA sequences to modify an organism’s genome locally or systematically. Core tools include CRISPR-Cas9, TALENs, and ZFNs.
- Scope:
- Site-specific editing of single or multiple genes (e.g., correcting disease-causing mutations, inserting functional genes).
- Epigenetic modifications (e.g., DNA methylation, histone editing).
- Optimization of regulatory elements (e.g., promoter/enhancer engineering).
- Goal: Optimize or repair biological functions by editing existing genomes.
- Synthetic Genomics
- Definition: The discipline of constructing artificial genomes or reengineering natural genomes through chemical synthesis and assembly of large DNA fragments (from chromosomes to entire genomes).
- Scope:
- De novo whole-genome synthesis (e.g., the JCVI-syn3.0 mycoplasma genome).
- Modular genome redesign and reprogramming (e.g., the yeast Sc2.0 project).
- Genome minimization and functional optimization (e.g., minimal genome studies).
- Goal: Explore the principles of life design and develop artificial life systems by creating new genomes.
II. Technical Approaches and Key Differences
Aspect | Genome Engineering | Synthetic Genomics |
---|---|---|
Scale | Local gene/epigenetic edits (<10 kb) | Whole-genome or chromosome-level (>1 Mb) |
Methodology | Editing and optimizing natural genomes | Full-cycle design from digital DNA to synthesis |
Toolchain | CRISPR, base editing, prime editing | CAD software, DNA synthesizers, yeast homologous recombination |
Technical Challenges | Off-target effects, delivery efficiency, multi-gene regulation | Long DNA synthesis errors, chromosome assembly/transplantation, functional validation |
Applications | Disease therapy, crop/industrial strain optimization | Artificial lifeforms, genome function analysis, biosafety chassis development |
Case Studies:
- Genome Engineering: CRISPR-Cas9 used in sickle cell anemia therapy (targeting HBB gene mutations).
- Synthetic Genomics: The 2010 JCVI-syn1.0 synthetic mycoplasma genome transplant demonstrated artificial genome-driven cellular activity.
III. Collaborative Evolution and Technological Synergy
- Toolchain Integration
- Synthetic Genomics relies on Genome Engineering: Chromosome transplantation requires gene editing tools (e.g., CRISPR) to remove host genomes.
- Genome Engineering benefits from Synthetic Tools: Synthetic regulatory elements (e.g., orthogonal promoters, insulators) improve editing precision.
- Data-Driven Design
- AI for Genome Design: Machine learning predicts gene network interactions (e.g., automated design in the Sc2.0 project), optimizing synthetic genome stability.
- Dynamic Validation: Single-cell multi-omics (RNA velocity, ATAC-seq) monitors real-time expression dynamics in edited or synthetic genomes.
- Standardization and Automation
- DBTL Cycle (Design-Build-Test-Learn): Synthetic genomics uses standardized gene libraries (BioBricks) and automated platforms (e.g., droplet microfluidics) for rapid iteration. Genome engineering employs high-throughput screening (e.g., MAGE) to optimize editing strategies.
IV. Key Challenges
Domain | Genome Engineering | Synthetic Genomics |
---|---|---|
Technical Hurdles | Low multi-gene regulation efficiency, transient epigenetic edits | High error rates in long DNA synthesis (>200 bp), low chromosome transplantation success (<5%) |
Ethics & Safety | Off-target carcinogenicity, irreversible ecological impacts | Biosafety concerns over artificial lifeforms, unpredictability of synthetic genomes |
Industrialization | High delivery system costs (AAV production), lengthy clinical approvals | DNA synthesis costs ($0.01/bp), lack of scalable chromosome assembly platforms |
Breakthroughs:
- Genome Engineering: Prime Editing 2.0 achieves DSB-free insertions with >50% efficiency.
- Synthetic Genomics: TdT enzyme-driven non-templated DNA synthesis surpasses 1 kb, reducing costs to $0.001/bp.
V. Future Synergy Directions
- Large-Scale Genome Engineering
- Hybrid Synthetic-Editing Strategies: Pre-synthesized functional modules (e.g., stress-resistant pathways) integrated via CRISPR-Cas12m for in vivo assembly in mammalian cells.
- Quantum Computing: Quantum annealing optimizes multi-target editing to minimize interference.
- In Vivo Genome Reprogramming
- In Situ DNA Synthesis: Phage Φ29 DNA polymerase synthesizes predefined sequences directly in cells, bypassing in vitro assembly.
- Epigenetic-Synthetic Synergy: Synthetic genomes with programmable regulators (e.g., CRISPR-dCas9-methyltransferase fusions) enable environment-responsive gene expression.
- Ethical and Regulatory Innovation
- Dynamic Risk Assessment: AI monitors synthetic genome evolution in hosts for early risk detection.
- Global Standards: ISO/TC 276 promotes international certification for synthetic genome validation.
VI. Conclusion and Outlook
Genome Engineering and Synthetic Genomics represent a paradigm shift from “editing nature” to “creating nature.” The former focuses on precise manipulation of existing life systems, while the latter redefines life’s foundational logic. Their convergence (e.g., hybrid synthetic-editing systems) will drive next-generation biomanufacturing platforms:
- Healthcare: Synthetic “ultra-safe” chassis (e.g., virus receptor-free human stem cells) combined with genome editing for organ regeneration.
- Industry: Modular synthetic chromosomes with orthogonal metabolic pathways, dynamically regulated via editing.
- Basic Science: Minimal genomes and synthetic evolution experiments reveal life’s origins and adaptation mechanisms.
Over the next decade, as DNA synthesis costs approach $0.0001/bp and AI-automated toolchains mature, the boundaries between these fields will blur, ushering in an era of “designed life.”
Data sourced from public references. For collaboration or domain inquiries, contact: chuanchuan810@gmail.com