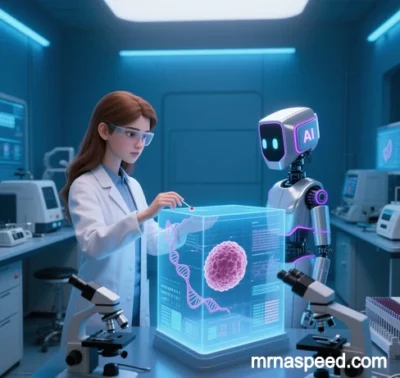
mRNA Velocity in Cancer: Advances in Transcription Rate Mutations and Therapeutic Prospects
mRNA velocity—defined as the elongation rate of RNA polymerase during transcription and the dynamic lifecycle of mRNA from synthesis to degradation—has emerged as a critical factor in cancer biology. Aberrant transcription rate mutations (e.g., accelerated or stalled elongation) in cancer-related genes drive tumorigenesis, metastasis, and drug resistance by altering mRNA splicing, stability, and translation efficiency. Below is a detailed analysis of molecular mechanisms, detection technologies, regulatory networks, therapeutic strategies, and future directions.
I. Molecular Mechanisms: How Transcription Rate Mutations Reshape Cancer Biology
1. Transcription Elongation and mRNA Isoform Diversity
Abnormal RNA polymerase II (Pol II) elongation rates in cancer promote alternative polyadenylation (APA) and splice variant generation. In breast cancer, transcriptional collisions between HMGXB3 and c-fms genes induce 3′-end polymorphisms, producing truncated or fused mRNAs that activate pro-oncogenic pathways like MAPK/ERK. Rate-dependent APA events enhance oncogene expression by altering mRNA stability and miRNA binding sites (e.g., loss of miR-21 targeting).
2. Transcription Rate-Epigenetic Crosstalk
Transcription rate mutations modify chromatin accessibility. In glioblastoma (GBM), rapid Pol II elongation enriches mutations near transcription start sites (TSS), disrupting tumor suppressor gene promoters (e.g., TP53) while activating oncogene super-enhancers (e.g., MYC). These mutations correlate with mRNA abundance, highlighting transcriptionally active regions as hotspots for genomic instability.
3. Ribosome-Pol II Coordination
In bladder cancer, ARID1A loss disrupts Pol II elongation-ribosome speed balance. ARID1A normally maintains ribosome progression to prevent mRNA translation errors; its absence stalls ribosomes on oncogenic mRNAs (e.g., CCND1), triggering the unfolded protein response (UPR) and tumor proliferation.
II. Detection Technologies: Multidimensional Profiling
1. RNA-Seq and Dynamic Transcriptomics
- Long-read sequencing (e.g., PacBio Iso-Seq) captures rate-dependent splicing events. In prostate cancer, LMTK2 splice isoforms (e.g., exon-skipping variants) correlate with Pol II elongation rates and predict metastasis risk.
- Single-cell RNA velocity (e.g., scVelo) infers cell-state transitions by comparing nascent (unspliced) and mature (spliced) mRNA ratios. In gliomas, this reveals MYC-driven transcription rate gradients linked to cancer stem cell differentiation.
2. Live-Cell Imaging and Real-Time Tracking
CRISPR-tagged MS2/MCP systems enable real-time visualization of Pol II elongation at oncogenic loci (e.g., EGFR). In lung cancer, EGFR mutations double Pol II elongation rates, accelerating oncogenic mRNA production while increasing splicing errors.
3. Spatial Transcriptomics and Chromatin Interactions
MERFISH combined with Hi-C data shows that transcription rates in ERα-positive breast cancer cells correlate with chromatin loop formation, driving aberrant enhancer-oncogene interactions (e.g., CCND1).
III. Regulatory Networks: RNA-Protein Interactions
1. RNA-Binding Proteins (RBPs)
RBPs like HNRNPA1 modulate Pol II elongation by binding nascent mRNA 5’UTRs. In colorectal cancer, HNRNPA1 phosphorylation reduces its binding capacity, stalling Pol II at APC loci and generating truncated oncogenic variants.
2. Non-Coding RNA Regulation
- miRNA Feedback: miR-34a slows oncogene elongation (e.g., BCL2) by targeting Pol II cofactors (e.g., CDK9), inducing apoptosis.
- ceRNA Networks: In liver cancer, lncRNA NEAT1 sponges miR-200c to derepress CDK12 (a Pol II elongation regulator), accelerating pro-metastatic gene (e.g., VEGFA) transcription.
3. tRNA Modification and Translation-Transcription Coupling
Mutations in tRNA modifiers (e.g., NSUN2) alter anticodon wobble, forcing ribosomes to slow translation of missense codons. This deceleration feeds back to Pol II via mechanostress signals, reducing oncogene transcription rates and promoting adaptive drug resistance.
IV. Therapeutic Strategies: Targeting Transcription Rates
1. Small-Molecule Inhibitors
- CDK9 Inhibitors (e.g., Atuveciclib) block Pol II phosphorylation to slow oncogene elongation (e.g., MYC), showing efficacy in diffuse large B-cell lymphoma.
- BET Inhibitors (e.g., JQ1) disrupt BRD4-Mediator interactions, reducing Pol II dwell time at super-enhancers to suppress tumor stemness.
2. RNA-Based Therapies
- Antisense Oligonucleotides (ASOs) correct oncogenic splicing (e.g., BRAF V600E), restoring normal elongation rates and shrinking melanoma tumors by 60% in preclinical models.
- CRISPR-Cas13d RNA Editing repairs splicing errors (e.g., EGFRvIII), restoring tumor-suppressive isoforms.
3. Combination Therapy Optimization
Dynamic pharmacodynamic models guide sequential dosing. In breast cancer, CDK4/6 inhibitors (e.g., Palbociclib) slow cell cycle-dependent transcription, synergizing with CDK9 inhibitors to induce apoptosis.
V. Challenges and Future Directions
1. Technical Limitations
- Single-Molecule Spatiotemporal Tracking: Current tools cannot simultaneously resolve Pol II elongation, splice factor recruitment, and nuclear export in real time.
- Heterogeneity Modeling: Tumor spatial heterogeneity (e.g., hypoxic cores vs. perivascular regions) requires integration with spatial metabolomics and AI.
2. Clinical Translation Barriers
- Off-Target Effects: CDK9 inhibitors may disrupt stress responses in normal cells, necessitating tumor-targeted delivery systems (e.g., LNP encapsulation with microenvironment-responsive release).
- Drug Resistance: Tumors evade rate inhibition via alternative kinase upregulation (e.g., CDK12) or intron retention, demanding multi-target therapies.
3. Emerging Frontiers
- Quantum Biology Tools: Gold nanoparticle-enhanced Raman spectroscopy non-invasively monitors Pol II conformational changes to predict rate mutations.
- Synthetic Biology Circuits: Engineered RNA polymerases (e.g., tunable T7 RNAP) enable precise control of oncogene transcription dynamics.
Conclusion
mRNA velocity adds a new dimension to cancer therapy, shifting focus from static gene sequences to dynamic transcription kinetics. Over the next decade, integrating single-molecule technologies, AI models, and RNA-targeted therapies could enable spatiotemporally precise modulation of transcription rates, advancing cancer treatment toward a “dynamic equilibrium” paradigm. As Nature Reviews Cancer states: “Understanding how transcription rates shape cancer genomes and phenotypes is key to overcoming tumor heterogeneity and drug resistance.”
Data sourced from publicly available references. For collaborations or domain inquiries, contact: chuanchuan810@gmail.com.